Body-Building - Vertebrate Development
John Merck
Growth and evolution:
Remember, ontogeny pertains to the individual, evolution to the lineage. (TV sci-fi plots notwithstanding.) That doesn't mean they aren't connected in any way. Now that we know something about the evolutionary pattern of Vertebrata, let's fill in their developmental pattern.
Heterchrony:
Subtle changes over evolutionary time in an organism's developmental timetable are a potent source of overall evolutionary change. This is an idea with a history as long as the study of evolution.- Development is a process of differentiation from generalized to specialized form, with general structures appearing first.
- The embryo of any creature is never identical to the adult of any other.
Ernst Haeckel (1836 - 1919) Noted that the embryos of evolutionarily derived creatures may pass through stages in which they display ancestral traits. The human embryo, for instance, at certain stages has a tail and open gill slits. Proposed what he termed the biogenic law which maintains that "ontogeny recapitulates phylogeny." He attempted to integrate it with Darwinian evolution through the notion that evolutionary change is accomplished by the addition of new changes to the end of the embryological or developmental sequence. This idea seems simplistic when you consider what it implies about the evolution of butterflies, but it was eagerly embraced for the first half of the 20th century. (Note: Haeckel was an interesting originator of vocabulary. He gave us the terms "heterochrony" and "biology."
Karl Ernst von Baer (1792 - 1876) - Was an opponent of recapitulationism and set forth a series of developmental laws that held that:
A survey of the evolution of vertebrate developmental pathways vindicates von Baer, as we progress from general to specific, but leave room for more detailed illumination. This was supplied by:
Stephen J. Gould: (1941 - 2002) Accumulating evidence during the 20th century revealed many weaknesses in the "biogenic law." Von Baer's laws, while holding up intact, cried out for elaboration. In 1977, Stephen Gould stepped to the plate with the terminology and organization of heterchrony as we understand it. We have two basic forms of heterochrony:
General patterns of heterochrony:
- Paedomorphosis: The adult of a derived organism resembles a juvenile of the ancestor. Compare the derived, paedomorphic axolotl to a "normal" relative, the frosted flat woods salamander. (Frosted flat woods salamander.)
- Peramorphosis: The juvenile of a derived organism resembles an adult of the ancestor. Compare the derived peramorphic veiled chameleon to a "normal" ancestral common chameleon.


Adult derived axolotl (left) resembles juvenile ancestral frosted flat woods salamander (right) from Wikimedia and Wikipedia
Eumetazoan Development:
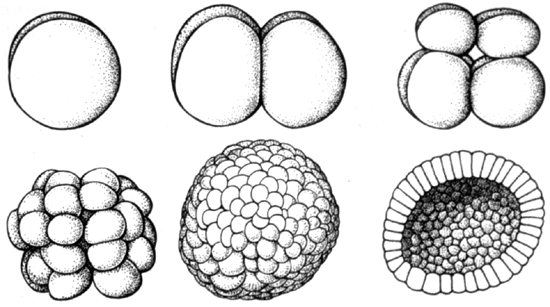
Cleavage from A. S. Romer. 1977. The Vertebrate Body.
Polarity: In all ova yolky material tends to concentrate at one end, yielding:
- "Animal" pole, the non-yolky side
- "Vegetal" pole, the yolky part.
Cleavage Phase of rapid cell division with little overall growth. The zygote transforms into a hollow sphere of cells, the blastula. The space in the middle is the blastocoel
The blastula has two cell types:
- Smaller cells nearer the "animal" pole.
- Larger, yolky cells nearer the "vegetal" pole.

Gastrulation from A. S. Romer. 1977. The Vertebrate Body.
- Cells of the "vegetal" hemisphere form a flat plate then invaginate inward, yielding a two layered hemisphere.
- The rim of this concavity quickly closes into a small opening, the blastopore.
- Endoderm: Destined to give rise to the gut and associated structures. The space enclosed by the endoderm is the archenteron, the precursor to the cut cavity.
- Ectoderm: Destined to give rise to the outer surface.
In cnidarians, the gastrula assumes the form of the planktonic planula larva which then directly develops features of an adult with no front, back, left, or right. For bilaterians, however, it is more complicated.
Bilaterian Development:
Mesoderm: As the illustration indicates, a third basic cell type, mesoderm, is present in the blastula, forming a collar around the large cells of the vegetal pole. They are fated to give rise to a third germ layer. Like the endoderm, they invaginate into the interior, but do so asymmetrically, extending along one side of the archenteron. This extension marks the animal's plane of bilateral symmetry. From there, mesoderm cells proliferate into the space between the endoderm and ectoderm, giving rise to a great range of three-dimensional structures and organs.The Coelom: A characteristic feature of bilaterians is the presence of a coelom or body cavity. This feature allows for:
- increased gut expansion,
- hydrostatic structure against which muscles may act, facilitating purposeful locomotion
- use of coelomic fluid in gas and nutrient transport, breaking dependence on simple diffusion and allowing larger body size.
The evolution of the coelom opened many vistas for animal evolution, including significantly expanded locomotor strategies. Cnidarians show the limits of what a hydrostatic skeleton can do for an animal with a single module. Bilaterians, however, display body segmentation in which separate modules of the hydrostatic skeleton can lengthen and shorten, facilitating much more complex movement. This makes possible activities like:
- Purposeful locomotion
- Burrowing into the substrate
Specialized organs: These capabilities came at a price. Animals with only endoderm and ectoderm don't need to worry about gas exchange and elimination of nitrogenous waste, because no living cell is so far from the body surface that simple diffusion can't do the trick. Bilaterians, in contrast, usually require specialized organs for functions like:
- Gas exchange
- Nitrogenous waste elimination
- Circulation
- Coordination of the nervous system
- Sensing the environment (useful to motivate and manage locomotion)
Deuterostome Development:
Bilateria breaks down into two major groups that were first distinguished by developmental characters (although molecular phylogenies have supported them):- Protostomia
- Deuterostomia
Protostomia: Includes arthropods, mollusks, annelid worms, brachiopods, and bryozoans plus some minor groups. Synapomorphies include:
- Blastopore gives rise directly to mouth and anus by elongating and "pinching off." (right - a)
- Development of the coelom is schizocoelomate, in which the coelom forms from direct cavitation of the mesoderm.
- Development is determinate, in which cell fates are absolute.
- Cleavage of embryonic cells is spiral, or arguably derived from a spiral pattern.
- Nerve cord is ventral to gut tube in adults.
Deuterostomia: Includes chordates and echinoderms plus some minor groups. Synapomorphies include:
- A distinct deuterostomous pattern of development (right - b) where the blastopore becomes the anus and the mouth develops from a secondary opening.
- Development of the coelom is enterocoelomate, in which the coelom originates as an out-pouching of the gut tube.
- Development is indeterminate development: i.e. the fate of a given cell is fluid and based on inductive relationships with neighboring cells and tissues.
- Cleavage of embryonic cells is radial , giving embryos the ability to twin.
- Nerve chord forms dorsal to gut tube in adults.
Yolk and cleavage:
So far our examples have been of animals whose ova contain relatively little yolk. In fact, among chordates yolk quantity can range from little to huge (consider a hen's egg, in which the yolk greatly outweighs the ovum's cytoplasm.) Because yolk is not metabolically active, its presence hinders cleavage, yielding distinct patterns:- Holoblastic: There is little yolk and cleavage is complete.
- Meroblastic: Enough yolk is present that cleavage is, to some degree, incomplete (A - C right).
Gastrulation in a meroblastic embryo occurs not at a distinct blastopore, but along a furrow called the primitive streak.
Chordate Development:
Neurulation: The indeterminate nature of deuterostome development, in which the fates of specific cells are influenced by inductive relationships with other cells, is illustrated by the next big step chordate development - the formation of the neural tube that gives rise to the central nervous system. In this process, the activity of mesoderm cells triggers a cascade of events.- Cells on the mid line of the mesoderm form a cylinder that becomes the notochord.
- Mesoderm to either side folds outward and expands down the side of the gastrula's inner surface.
- An inductive relationship causes the ectoderm directly above the notochord to sink downward, forming a trough, and ectoderm to either side to rise up forming crests to either side. The trough collapses into a hollow tube lying above the precursor to the notochord. This is the neural tube - the precursor to the brain and spinal cord.
Stupid mesoderm tricks: While neurulation is happening, mesoderm is also busy. Aside from the gut tube (endoderm), skin, and nervous system (ectoderm), most of the body derives from mesoderm. Here are a few major features:
- Intermediate and Lateral Plate Mesoderm: Mesoderm extending down the sides of the embryo in which the coelom forms coalesces into zones with distinct fates:
- Paraxial mesoderm gives rise to somites (see below).
- Intermediate mesoderm gives rise to kidneys and gonads.
- Lateral plate mesoderm gives rise to the coelom, body wall, and mesentaries (sheets of tissue connecting gut tube - suspended within the coelom - to the body wall).
- Somites: Dorsal to (above) the lateral plate, rectangular pouches of mesoderm bud off to form little hollow capsules on either side of the notochord. These are the somites - precursors to body segments from which dermis, muscles, and much of the skeleton forms. The first one forms near the front of the embryo, with successive somites budding off behind it. Animation of zebra-fish somite development.
- Somites give rise to three precursor tissues with distinct fates:
- Dermatome - dermis of skin
- Myotome - striated muscle
- Sclerotome - axial skeleton
- Mesenchyme: Mesoderm gives rise to a network of star-shaped cells capable of amoeboid movement. These are the mesenchyme cells - the connective tissue of the embryo. They give rise to the circulatory system and (in vertebrates) most of the skeleton. We will meet them again.
Vertebrate Development:
In some ways, Branchiostoma is distinctly lacking. It has no:- Head (or space to put one)
- Special sense organs
- Skeleton
- Peripheral nervous system
Neural Crest: Beside endoderm, mesoderm, and ectoderm, vertebrates have a fourth major tissue type, neural crest. To understand it, consider a vertebrate twist on neural tube formation. Among vertebrates, neural tube formation progresses as in other chordates, but with one difference: As the neural tube forms, masses of ectodermal cells break away from the crests to either side of the neural fold and aggregate as neural crest cells. Some of these give rise to the peripheral nervous system. Others migrate to various locations in the body, giving rise to:
- Skin pigment (in urochordates this is all they do)
- The anterior (front) skeleton of the head
- The skeleton of the gill arches and jaws
- Specialized cells of the adrenal and pituitary glands
- Dental papillae (primordia of the pulp cavity and dentine)

Inductive formation of the craniate eye from
Anatomical Foundations of Neuroscience - University of Western Ontario
Note: many other structures, including:
- teeth
- scales
- feathers
- fur
Ectoderm inventory: We have now discussed four distinct types of ectoderm:
- "Normal" exterior ectoderm
- Neural tube ectoderm
- Neural crest ectoderm
- Ectodermal placodes
Origin of the head:
Beyond neural crest, what do all of these taxa have in common that the outgroups (Urochordata and Cephalochordata) lacks? Heads - a radical departure. (Note: the front end of Pikaia may be the functional equivalent of a vertebrate head, but doesn't resemble it anatomically and sports dissimilar sense organs.The appearance of the head is one of the major enigmas of chordate evolution because it is so sudden. No fossil chordate appears to have a partial head. In fossil non-craniate deuterostomes like Yunnanozoon, and vetulicolians, the front of the body is occupied by a mouth and pharynx with no brain or recognizable special sense organs, or even room to put them. In craniates, the head is present in front of it, and contains the special sense capsules. Is there a connection between heads and neural crest, which appears at the same point in evolution?
First, a digression.
Regulatory genes
The structure of DNA was discovered in 1953, and its role as the physical repository of genes illuminated in the following decades. How information encoded as nucleic acid is expressed as proteins is well-known.
The magic of protein synthesis is that DNA is only transcribed into mRNA when the protein it codes for is needed. E.G.: The bacterium Escherichia coli freely metabolizes glucose, but if glucose is lacking, and lactose is present, it can metabolize it by producing an enzyme, beta-galactosidase, that breaks lactose down in to glucose and galactose. But how does it know when to make beta-galactosidase?
The work of François Jacob and Jacques Monod established the answer (Nobel Prize in 1965): Adjacent to the gene for beta-galactosidase is a small gene to which a protein, the lac repressor binds. This blocks RNA polymerase from unzipping the DNA and making mRNA. But, lactose, itself, binds to the lac repressor causing it to fall off of the DNA strand and allowing RNA polymerase to do its thing. Once the lactose has been metabolized, the lac repressor reattaches and transcription ceases. Animation.
Of course, the lac repressor protein is coded for by a repressor gene whose activity may be regulated by other repressors. Indeed, the expression of genes as proteins is the result of complex interactions of regulatory and structural genes and their protein products.
A similar system appears to govern the differentiation of regions of the body.
HOX genes
Hox genes: During the late 20th century it became known that segmentation in bilaterians is governed by a special class of regulatory genes. The story:
Fruit flies are a favorite model for geneticists, with short generation spans and interesting mutations that often effect entire sections of the body (modifying or eliminating body segments and/or the appendages that grow from them). Investigation into these segmentation-altering mutations revealed that they can be caused by mutations to eight genes. What makes this interesting:
- The genes are close to one another on a single chromosome, and are physically lined up in the same order as the segments of the body they effect.
- Each gene (of ~ 1000 base pairs) contains a nearly identical sequence of about 60 base-pairs called homeoboxes.
But it gets better: The search for homologs to fruit fly Hox genes found them in almost every animal surveyed. Mammals, for example, have four clusters of Hox gene homologs, in each of which the genes occur in the same order on the chromosome as the regions for which they code.
This is huge for two reasons:
- The fruit flies and mammals belong to the two major clades of bilaterians, and their last common ancestor lived over 600 mya, and yet they share important regions of the genome.
- The regions of the body in which paralogous genes are expressed allow us to establish their homologies.
Why do the numbers of Hox genes and Hox gene clusters differ? - Errors in transcriptions result in duplications in which paralogous copies of the genes are generated - i.e. genes coexisting within the same genome that trace their descent to a common ancestor genes.
What happens to an organisms phenotype (physical form) when a gene or gene cluster that controls body segmentation is duplicated?
Remember, Hox genes control segmentation and fundamental orientation of embryo. They are conservative gene clusters found throughout the animal realm. Besides controlling orientation and segmentation, each gene influences a specific region of the body.
Gene transcription errors have profound effects. One common consequence of such errors is the duplication of genes, where two paralogous copies of a gene are generated. (As opposed to homologous versions of physical structures. ) Once present, they can each evolve independently and ultimately code for different proteins.
Putting it together: In 2008, the genome of Branchiostoma was sequenced. We now know:
- The paralogs of the genes that are expressed in craniate neural crest cells are present in Branchiostoma (although the cells are non-migratory) and urochordates (where they migrate to form pigmentation cells.) Neural crest tissues are coded for by homeobox genes in craniates.
- The homeobox genes of craniates differ from those of Branchiostoma in an important respect: Rather than having one homeobox gene cluster, craniates all have at least two, arguably the result of a gene duplication event.
- The upshot is that the sudden appearance of a complex head and branchial skeleton may have been the result of Hox gene duplication and the subsequent independent evolution of the resulting gene clusters. This introduces an interesting variation on the traditional gradualist view of evolution. Quite possibly, the material that natural selection shaped into the head appeared all at once, as the result of a one-time occurance - the duplication of a group of genes.
Additional reading:
- Carroll, Sean. 2006. Endless Forms Most Beautiful: The New Science of Evo Devo W. W. Norton & Company.
- Stephen H. Gould. 1977. Ontogeny and Phylogeny Belknap Press.
- Lacalli, Thurston. 2024. The Cambrian fossil Pikaia, and the origin of chordate somites EvoDevo. Feb., 2024
- Romer, Alfred S. 1977. The Vertebrate Body W.B. Saunders Company.
- Michael Schubert, Hector Escriva, José Xavier-Neto, Vincent Laudet. 2006. Amphioxus and tunicates as evolutionary model systems. Trends in Ecology and Evolution. 21-5, 269-277.
- Stephen H. Gould. 1977. Ontogeny and Phylogeny Belknap Press.